Dual-Use Risks of Oncolytic Virus Engineering
Certain biotechnologies have the potential to facilitate pathogen engineering, increasing the risk of large-scale biological events, e.g., major pandemics. This article assesses the dual-use potential of viral engineering using the example of oncolytic viruses. It also offers recommendations for managing the biosecurity risks associated with this research.
Despite recent therapeutic advances, cancer remains a significant health concern. According to the World Health Organization (WHO), in 2022, there were an estimated 20 million new cancer cases and 9.7 million deaths.2 According to the most recent estimate, there were 53.5 million individuals alive who had received a cancer diagnosis in the past five years. About 1 in 5 people develop cancer in their lifetime, and approximately 1 in 9 men and 1 in 12 women die from the disease. Novel approaches to cancer therapeutics attract significant government funding and commercial attention.3 One line of cancer therapy that has evolved over recent years is the use of oncolytic viruses (OVs). These are a group of replicating viruses that preferentially infect and kill cancerous cells.4 The use of OVs has rapidly expanded over the past ten years and oncolytic viruses are now considered an important cancer immunotherapy method.5 Prior to 2010, only 15 clinical studies using oncolytic viruses had been registered on the ClinicalTrials.gov database. By March 2024, 188 studies had been registered.6
The origins of this line of therapy lie in the observation that tumors shrink after a natural viral infection. While in some instances, viruses infect and kill cancerous cells without harming normal tissue, natural viruses have shown limited efficacy as therapeutics.7 This has led to rapid expansion of research based on modifying oncolytic viruses genetically to change their host range or specificity, enhance immune responses targeting cancerous cells, and increase their lytic potential. For example, oncolytic adenoviruses can be engineered to express proteins that enhance apoptosis, a form of regulated cell death.8
While viral engineering may be crucial for developing effective therapeutics, this accelerated research involving genetic modification of oncolytic viruses can also pose risks. Researchers have previously raised the concern that viral engineering advances may enable the development of engineered pathogens for malicious misuse. Dual-use risks have previously been identified in viral vector research, which involves the use of viruses as carriers for the delivery of substances to target cells.9 When pursuing this line of research, scientists should base their choice of viruses to engineer on a well-founded assessment of their efficacy, safety, and dual-use potential. Thus, we aim to carefully examine the dual-use risks associated with oncolytic virus engineering with a view to aiding in this assessment. We identify high- and low-risk approaches and conclude with recommendations regarding the most appropriate strategies for creating oncolytic virus therapeutics, in light of these risks.
Choice of Virus for Oncolytic Virotherapy
The dual-use potential of oncolytic virus research depends on how directly viral engineering insights may be applied to pathogens. Thus, the choice of significantly influences dual-use risk. In contrast to other therapeutic applications of viruses, for instance for gene therapy, there is a particularly high chance of oncolytic virus research involving viruses related to human pathogens. An effective oncolytic virus can replicate in humans, have cancer-killing activity, and induce immune responses that can kill bystander tumor cells. Thus, among the ten virus families explored in clinical trials of oncolytic virotherapy are high-risk viruses such as the influenza, measles, and variola viruses (see Table 1). Influenza and measles viruses are both human pathogens against which a significant share of the population is immunized. Insights into the enhancement of these viruses, especially relating to the property of evading vital immune responses, could pose significant public health risks.
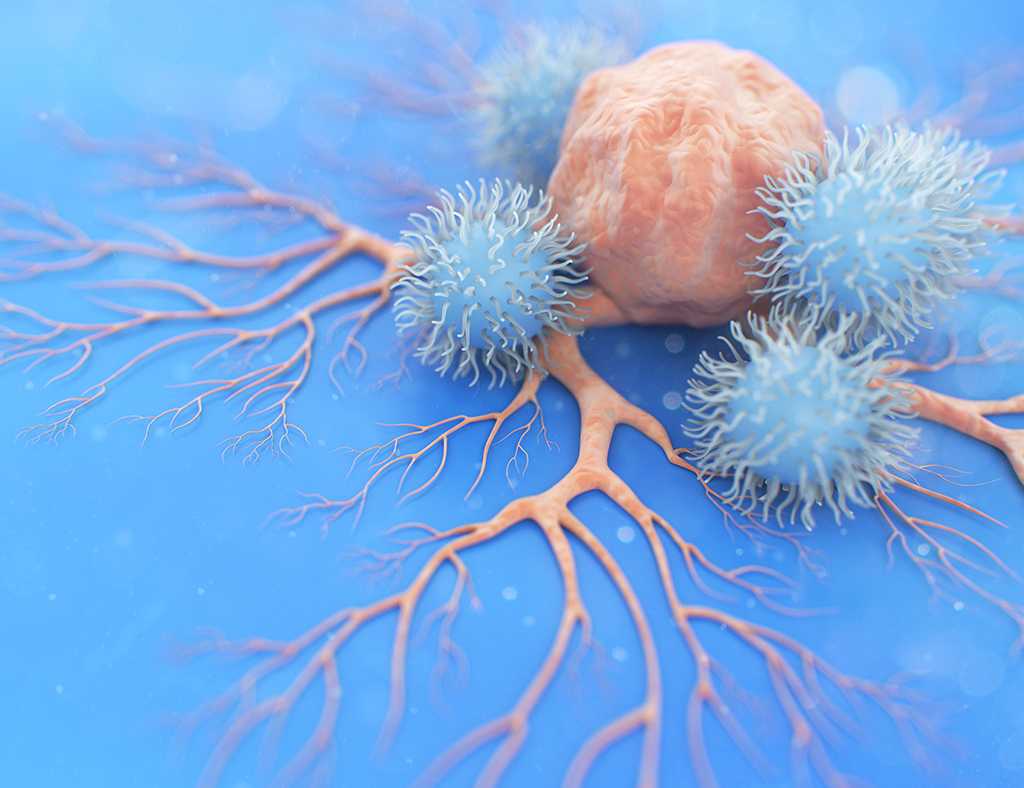
Similarly, multiple species of vaccinia viruses are used in this field since they feature significant benefits, such as efficient delivery, intravenous stability, large cloning capacity, and verified ability to induce efficient immune responses. Vaccinia belongs to the poxviridae family of viruses and is thus related to the variola virus, the agent that causes smallpox. In 2018, when a description of the creation of horsepox virus was published, biosecurity experts raised the concern that work on poxviruses such as vaccinia and horsepox may result in the dissemination of the skills required for the recreation of smallpox. Similarly, work on oncolytic viruses based on the vaccinia virus may also lower the barrier to misuse.
Level of risk of oncolytic virus | Properties | Examples |
---|---|---|
High risk | Related to potential pandemic pathogens | Orthomyxoviridae (Influenza10), Paramyxoviridae (Measles11), NDV12, Poxviridae (Vaccinia12), Variola13, Retroviridae14 |
Medium risk | Related to human or animal viruses with high pathogenicity | Picornaviridae (Polio15), SVV16, Retroviridae17, Rhabdoviridae (VSV18), Togaviridae19 |
Low risk | Related to human or animal viruses with low pathogenicity | Adenoviridae20, Herpesviridae (HSV-121), Parvoviridae22, Reoviridae23 |
Lowest risk | Incapable of unassisted human-to-human transmission | Adeno-associated viruses (AAV)24 |
Table 1. Characterization of Viruses Currently Used in Oncolytic Virus Research.
Medium-risk viruses include those related to human or animal viruses with high pathogenicity, while viruses related to low-pathogenicity agents generally pose low risks (see Table 1). The lowest risk are viruses that are incapable of unassisted human-to-human transmission, such as adeno-associated viruses or those known as vector-borne viruses, which are carried and spread by bites from mosquitoes, ticks, and fleas.
Risks from Developing Transferable Methods of Immune Evasion
Preexisting neutralizing antibodies can reduce the effective of an oncolytic virus, thus blunting its therapeutic effect. To prevent an undesired reduction in therapeutic efficacy, researchers engineer viruses to modulate the induction of host immune responses (i.e., immune evasion). Several methods have been developed to mask viral surface proteins with polymeric materials in order to enhance protection against neutralizing antibodies and extend the half-life of viral circulation.
For instance, adenoviruses and herpes simplex virus are frequently neutralized either by natural or vaccine-induced immunity. Adenovirus serotype 5 (Ad5) is significantly limited by preexisting neutralizing antibodies, a limitation that can be circumvented by replacing the hypervariable regions (HVRs) with versions that have less preexisting immunity.25 A similar approach could be used to create an adenovirus able to evade natural or vaccine-induced immunity.
Heritable strategies, such as permanently altering the virus genome for immune evasion, which will then be carried on by virus progeny, feature significant dual-use risks when they are transferable to agents with a pandemic potential.
Researchers are increasingly creating general viral engineering tools to modify several characteristics of viruses at once, making these capabilities more accessible to those without extensive virological expertise. General-purpose viral engineering methodologies that enable precise experiments could significantly lower the barrier to generating novel pandemic agents. More laboratories are using computational methods to predict critical loci, e.g., for .26 For instance, Ogden et al. have used data from an AAV (Adeno-associated viruses) to train models for optimizing several properties at once, including virus production, immune evasion, thermostability, and distribution.27
To evade immune responses to viral vectors and , researchers are engineering these vectors to modulate the induction of host immune responses through the insertion of distinct genetic elements, as discussed elsewhere.28 These universal elements for immune modulation may be particularly concerning as they are translatable to any virus with sufficient genomic flexibility.
Dual-Use Insights from Expanding Viral Tropism and Enhancing Virulence
An additional line of research with dual-use concerns is engineering the expansion of viral tropism—increasing the types of cells that a particular virus is capable of infecting. Dense Stroma can limit viral spread, replication, and infectivity. In light of this, several research groups are working on modifying oncolytic viruses to optimize their ability to target specific stromal components, such as cancer-associated fibroblasts (CAFs). The latter carry specific cell surface markers, fibroblast activation proteins (FAPs)29 that are upregulated in many tumors but also expressed on normal fibroblasts of several connective tissues. Oncolytic viruses (e.g., enadenotucirev, a oncolytic adenovirus) are being engineered to carry such FAP-specific fusion proteins.30 Even without malicious intent, viruses engineered for these properties may carry risks. There is potential for genetic recombination between vaccine vector viruses carrying engineered virulence elements and wildtype viruses,31 such as between oncolytic adenoviruses and wildtype adenoviruses. This may result in unforeseen enhancement of certain pathogens with regard to properties such as virulence and immune evasion.
Risks from Creating “Plug and Play” Approaches to Engineering Viral Properties
Synthetic biology researchers are proposing the development of “programmable” oncolytic viruses—viruses that are easily designed and programmed for specific purposes.32 Directed evolution platforms have been developed to create oncolytic viruses specific to certain cell surface receptors.33
Reverse genetics systems are available for essentially all virus families, sometimes with open access step-by-step protocols,34 setting the stage for a future where adding or eliminating synthetic or naturally occurring genetic elements and functions to or from a desired virus can be done with relative ease.35 Machine learning-based tools attempt to predict viral infectivity in a given host, and these methods may be highly transferable to other viral families.36 While these early studies are unlikely to represent a major risk in and of themselves, they are demonstrative of a trend toward using publicly available data and transferable machine learning tools to design and predict the infectivity of viruses with great accuracy.
In a similar vein, generating general protocols and platforms for viral engineering is associated with the generation of knowledge of viral properties and their modification.37 Detailed, publicly accessible protocols pose particular risks under certain circumstances38 and “enable researchers from different research backgrounds to master the use of the reverse genetic system”.39
Discussion: Mitigating Dual-Use Risks
Dual-use risks are emerging from new lines of research within the field of oncolytic viral therapy. While these advancements hold significant potential for improving human health, they also create opportunities for the development of biological weapons. The following discussion and recommendations are speculative in nature, as cancer researchers engaged in oncolytic virus studies have a deeper expertise in this area and well-founded rationales for their decisions. Nonetheless, researchers in this field should carefully assess the risks and explore the opportunities for mitigation.
Dual-use risks are emerging from new lines of research within the field of oncolytic viral therapy. Researchers should carefully assess the risks and explore the opportunities for mitigation.
The most straightforward way to reduce the risks from oncolytic virus therapy would be to work with viruses that are not related to potential pandemic pathogens. Researchers exploring oncolytic viruses that encounter inhibition due to preexisting immunity might opt for nonheritable methods of achieving immune evasion. For example, chemical modifications of adenoviruses have shown utility for immune evasion.40 An alternative to nonheritable enhancement methods is an increasing focus on administering immune-modulating agents pharmacologically to allow oncolytic viruses to circumvent innate antiviral immune responses.41 The use of carrier cells—delivery vehicles that protect viruses from neutralization—is another lower-risk method of improving oncolytic virus delivery to tissues.42
If different strategies feature similar benefits without the risks described above, cancer therapy strategies other than oncolytic viruses may be preferentially developed. In their latest annual review, the American Society of Clinical Oncology identified immune checkpoint inhibitors and cellular immunotherapies as the most promising advances in immunotherapy, while oncolytic virotherapy is not mentioned.43 Advances in molecular profiling now allow for highly targeted, tumor-specific antibody therapies. Chimeric antigen receptor T (CAR-T)44 cell therapies are also rapidly proving effective and being approved for various forms of lymphoma and other malignancies. Preferentially advancing these promising methods could create effective cancer therapies without the same level of dual-use risk as efforts to develop oncolytic viruses.
Footnotes
-
Acknowledgments: J. Sandbrink, PhD and J. Pannu, MD provided important feedback on earlier versions of the paper. The final version was also commented on by Prof. Kathryn Nixdorff. ↩
-
WHO. (2024, February 1). Global cancer burden growing, amidst mounting need for services. https://www.who.int/news/item/01-02-2024-global-cancer-burden-growing--amidst-mounting-need-for-services ↩
-
The White House. (2022, February 2). Fact Sheet: President Biden Reignites Cancer Moonshot to End Cancer as We Know It [Online post]. https://www.whitehouse.gov/briefing-room/statements-releases/2022/02/02/fact-sheet-president-biden-reignites-cancer- moonshot-to-end-cancer-as-we-know-it/ ↩
-
Patel, M. R., & Kratzke, R. A. (2013). Oncolytic virus therapy for cancer: The first wave of translational clinical trials. Translational Research, 161(4), 355–364. https://doi.org/10.1016/j.trsl.2012.12.010; Russell, S. J., Peng, K.-W., & Bell, J. C. (2012). Oncolytic virotherapy. Nature Biotechnology, 30(7), 658–670. https://doi.org/10.1038/nbt.2287 ↩
-
Russell, S. J., Bell, J. C., Engeland, C. E., & McFadden, G. (2022). Advances in oncolytic virotherapy. Communications Medicine, 2(1), 33. https://doi.org/10.1038/s43856-022-00098-4 ↩
-
National Library of Medicine. (2024, March 1). ClinicalTrials.Gov. https://clinicaltrials.gov ↩
-
Asada, T. (1974). Treatment of human cancer with mumps virus. Cancer, 34(6), 1907–1928. https://doi.org/10.1002/1097-0142(197412)34:6...; Patel & Kratzke, 2013; Russell et al., 2012. ↩
-
Yoon, A.-R., Hong, J., & Yun, C.-O. (2017). Adenovirus-mediated decorin expression induces cancer cell death through activation of p53 and mitochondrial apoptosis. Oncotarget, 8(44), 76666–76685. https://doi.org/10.18632/oncotarget.20800 ↩
-
Sandbrink, J. B., Alley, E. C., Watson, M. C., Koblentz, G. D., & Esvelt, K. M. (2022). Insidious Insights: Implications of viral vector engineering for pathogen enhancement. Gene Therapy. https://doi.org/10.1038/s41434-021-00312-3; Sandbrink, J. B., & Koblentz, G. D. (2022). Biosecurity risks associated with vaccine platform technologies. Vaccine, 40(17), 2514–2523. https://doi.org/10.1016/j.vaccine.2021.02.023 ↩
-
Yang, H., Lei, G., Sun, F., Cheng, J., Yan, J., Zhang, S., & Yang, P. (2022). Oncolytic Activity of a Chimeric Influenza A Virus Carrying a Human CTLA4 Antibody in Hepatocellular Carcinoma. Frontiers in Oncology, 12, 875525. https://doi.org/10.3389/fonc.2022.875525 ↩
-
Msaouel, P., Opyrchal, M., Dispenzieri, A., Peng, K. W., Federspiel, M. J., Russell, S. J., & Galanis, E. (2018). Clinical Trials with Oncolytic Measles Virus: Current Status and Future Prospects. Current Cancer Drug Targets, 18(2), 177–187. https://doi.org/10.2174/1568009617666170222125035 ↩
-
Heo, J., Reid, T., Ruo, L., Breitbach, C. J., Rose, S., Bloomston, M., Cho, M., Lim, H. Y., Chung, H. C., Kim, C. W., Burke, J., Lencioni, R., Hickman, T., Moon, A., Lee, Y. S., Kim, M. K., Daneshmand, M., Dubois, K., Longpre, L., … Kirn, D. H. (2013). Randomized dose-finding clinical trial of oncolytic immunotherapeutic vaccinia JX-594 in liver cancer. Nature Medicine, 19(3), 329–336. https://doi.org/10.1038/nm.3089 ↩
-
Ricordel, M., Foloppe, J., Pichon, C., Sfrontato, N., Antoine, D., Tosch, C., Cochin, S., Cordier, P., Quemeneur, E., Camus-Bouclainville, C., Bertagnoli, S., & Erbs, P. (2017). Cowpox Virus: A New and Armed Oncolytic Poxvirus. Molecular Therapy - Oncolytics, 7, 1–11. https://doi.org/10.1016/j.omto.2017.08.003 ↩
-
Li, F., Guo, Y., Han, L., Duan, Y., Fang, F., Niu, S., Ba, Q., Zhu, H., Kong, F., Lin, C., & Wen, X. (2012). In vitro and in vivo growth inhibi- tion of drug-resistant ovarian carcinoma cells using a combination of cisplatin and a TRAIL-encoding retrovirus. Oncology Letters, 4(6), 1254–1258. https://doi.org/10.3892/ol.2012.926 ↩
-
Toyoda, H., Ido, M., Hayashi, T., Gabazza, E. C., Suzuki, K., Kisenge, R. R., Kang, J., Hori, H., & Komada, Y. (2004). Experimental treatment of human neuroblastoma using live-attenuated poliovirus. International Journal of Oncology, 24(1), 49–58. ↩
-
Reddy, P. S., Burroughs, K. D., Hales, L. M., Ganesh, S., Jones, B. H., Idamakanti, N., Hay, C., Li, S. S., Skele, K. L., Vasko, A.-J., Yang, J., Watkins, D. N., Rudin, C. M., & Hallenbeck, P. L. (2007). Seneca Valley Virus, a Systemically Deliverable Oncolytic Picornavirus, and the Treatment of Neuroendocrine Cancers. JNCI: Journal of the National Cancer Institute, 99(21), 1623–1633. https://doi.org/10.1093/jnci/djm198 ↩
-
Tai, C.-K. (2012). Replicating retroviral vectors for oncolytic virotherapy of experimental hepatocellular carcinoma. Oncology Reports. https://doi.org/10.3892/or.2012.1789 ↩
-
Muik, A., Dold, C., Geiß, Y., Volk, A., Werbizki, M., Dietrich, U., & von Laer, D. (2012). Semireplication-competent vesicular stomatitis virus as a novel platform for oncolytic virotherapy. Journal of Molecular Medicine, 90(8), 959–970. https://doi.org/10.1007/s00109-012-0863-6 ↩
-
Cai, J., & Yan, G. (2021). The Identification and Development of a Novel Oncolytic Virus: Alphavirus M1. Human Gene Therapy, 32(3–4), 138–149. https://doi.org/10.1089/hum.2020.271 ↩
-
Kirn, D. (2000). Replication-selective oncolytic adenoviruses: Virotherapy aimed at genetic targets in cancer. Oncogene, 19(56), 6660–6669. https://doi.org/10.1038/sj.onc.1204094; Zhao, Y., Liu, Z., Li, L., Wu, J., Zhang, H., Zhang, H., Lei, T., & Xu, B. (2021). Oncolytic Adenovirus: Prospects for Cancer Immunotherapy. Frontiers in Microbiology, 12, 707290. https://doi.org/10.3389/fmicb.2021.707290 ↩
-
Harrington, K. J., Puzanov, I., Hecht, J. R., Hodi, F. S., Szabo, Z., Murugappan, S., & Kaufman, H. L. (2015). Clinical development of talimogene laherparepvec (T-VEC): A modified herpes simplex virus type-1–derived oncolytic immunotherapy. Expert Review of Anticancer Therapy, 15(12), 1389–1403. https://doi.org/10.1586/14737140.2015.1115725; Masoud, S. J., Hu, J. B., Beasley, G. M., Stewart, J. H., & Mosca, P. J. (2019). Efficacy of Talimogene Laherparepvec (T-VEC) Therapy in Patients with In-Transit Melanoma Metastasis Decreases with Increasing Lesion Size. Annals of Surgical Oncology, 26(13), 4633–4641. https://doi.org/10.1245/s10434-019-07691-3 ↩
-
Marchini, A., Bonifati, S., Scott, E. M., Angelova, A. L., & Rommelaere, J. (2015). Oncolytic parvoviruses: From basic virology to clinical applications. Virology Journal, 12(1), 6. https://doi.org/10.1186/s12985-014-0223-y ↩
-
Samson, A., Scott, K. J., Taggart, D., West, E. J., Wilson, E., Nuovo, G. J., Thomson, S., Corns, R., Mathew, R. K., Fuller, M. J., Kottke, T. J., Thompson, J. M., Ilett, E. J., Cockle, J. V., van Hille, P., Sivakumar, G., Polson, E. S., Turnbull, S. J., Appleton, E. S., … Melcher, A. A. (2018). Intravenous delivery of oncolytic reovirus to brain tumor patients immunologically primes for subsequent checkpoint blockade. Science Translational Medicine, 10(422), eaam7577. https://doi.org/10.1126/scitranslmed.aam7577 ↩
-
Pan, J. G., Zhou, X., Zeng, G. W., & Han, R. F. (2012). Potent antitumour activity of the combination of HSV‐TK and endostatin armed oncolytic adeno‐associated virus for bladder cancer in vitro and in vivo. Journal of Surgical Oncology, 105(3), 249–257. https://doi.org/10.1002/jso.22107 ↩
-
Roberts, D. M., Nanda, A., Havenga, M. J. E., Abbink, P., Lynch, D. M., Ewald, B. A., Liu, J., Thorner, A. R., Swanson, P. E., Gorgone, D. A., Lifton, M. A., Lemckert, A. A. C., Holterman, L., Chen, B., Dilraj, A., Carville, A., Mansfield, K. G., Goudsmit, J., & Barouch, D. H. (2006). Hexon-chimaeric adenovirus serotype 5 vectors circumvent pre-existing anti-vector immunity. Nature, 441(7090), 239–243. https://doi.org/10.1038/nature04721 ↩
-
Marques, A. D., Kummer, M., Kondratov, O., Banerjee, A., Moskalenko, O., & Zolotukhin, S. (2021). Applying machine learning to predict viral assembly for adeno-associated virus capsid libraries. Molecular Therapy - Methods & Clinical Development, 20, 276–286. https://doi.org/10.1016/j.omtm.2020.11.017 ↩
-
Ogden, P. J., Kelsic, E. D., Sinai, S., & Church, G. M. (2019). Comprehensive AAV capsid fitness landscape reveals a viral gene and enables machine-guided design. Science, 366(6469), 1139–1143. https://doi.org/10.1126/science.aaw2900 ↩
-
Sandbrink, J. B., Alley, E. C., Watson, M. C., Koblentz, G. D., & Esvelt, K. M. (2022). Insidious Insights: Implications of viral vector engineering for pathogen enhancement. Gene Therapy. https://doi.org/10.1038/s41434-021-00312-3 ↩
-
Rettig, W. J., Chesa, P. G., Beresford, H. R., Feickert, H.-J., Jennings, M. T., Cohen, J., Oettgen, H. F., & Old, L. J. (1986). Differential expression of cell surface antigens and glial fibrillary acidic protein in human astrocytoma subsets. Cancer Research, 46(12_Part_1), 6406–6412. ↩
-
Freedman, J. D., Duffy, M. R., Lei-Rossmann, J., Muntzer, A., Scott, E. M., Hagel, J., Campo, L., Bryant, R. J., Verrill, C., Lambert, A., Miller, P., Champion, B. R., Seymour, L. W., & Fisher, K. D. (2018). An Oncolytic Virus Expressing a T-cell Engager Simultaneously Targets Cancer and Immunosuppressive Stromal Cells. Cancer Research, 78(24), 6852–6865. https://doi.org/10.1158/0008-5472. CAN-18-1750 ↩
-
Baldo, A., Leunda, A., Willemarck, N., & Pauwels, K. (2021). Environmental Risk Assessment of Recombinant Viral Vector Vaccines against SARS-Cov-2. Vaccines, 9(5), 453. https://doi.org/10.3390/vaccines9050453 ↩
-
Monie, D. D., Bhandarkar, A. R., Parney, I. F., Correia, C., Sarkaria, J. N., Vile, R. G., & Li, H. (2021). Synthetic and systems biology principles in the design of programmable oncolytic virus immunotherapies for glioblastoma. Neurosurgical Focus, 50(2), E10. https://doi.org/10.3171/2020.12.FOCUS20855; Twumasi-Boateng, K., Pettigrew, J. L., Kwok, Y. Y. E., Bell, J. C., & Nelson, B. H. (2018). Oncolytic viruses as engineering platforms for combination immunotherapy. Nature Reviews Cancer, 18(7), 419–432. https://doi.org/10.1038/s41568-018-0009-4 ↩
-
Dai, H.-S., Liu, Z., Jiang, W., & Kuhn, R. J. (2013). Directed Evolution of a Virus Exclusively Utilizing Human Epidermal Growth Factor Receptor as the Entry Receptor. Journal of Virology, 87(20), 11231–11243. https://doi.org/10.1128/JVI.01054-13 ↩
-
Pannu, J., Sandbrink, J. B., Watson, M., Palmer, M. J., & Relman, D. A. (2022). Protocols and risks: When less is more. Nature Protocols, 17(1), 1–2. https://doi.org/10.1038/s41596-021-00655-6; Xie, X., Lokugamage, K. G., Zhang, X., Vu, M. N., Muruato, A.E., Menachery, V. D., & Shi, P.-Y. (2021). Engineering SARS-CoV-2 using a reverse genetic system. Nature Protocols, 16(3), 1761–1784. https://doi.org/10.1038/s41596-021-00491-8 ↩
-
Maroun, J., Muñoz-Alía, M., Ammayappan, A., Schulze, A., Peng, K.-W., & Russell, S. (2017). Designing and building oncolytic viruses. Future Virology, 12(4), 193–213. https://doi.org/10.2217/fvl-2016-0129 ↩
-
Karabulut, O. C., Karpuzcu, B. A., Türk, E., Ibrahim, A. H., & Süzek, B. E. (2021). ML-AdVInfect: A Machine-Learning Based Adenoviral Infection Predictor. Frontiers in Molecular Biosciences, 8, 647424. https://doi.org/10.3389/fmolb.2021.647424 ↩
-
Sandbrink, J. B., & Koblentz, G. D. (2022). Biosecurity risks associated with vaccine platform technologies. Vaccine, 40(17), 2514–2523. https://doi.org/10.1016/j.vaccine.2021.02.023 ↩
-
Pannu, J., Sandbrink, J. B., Watson, M., Palmer, M. J., & Relman, D. A. (2022). Protocols and risks: When less is more. Nature Protocols, 17(1), 1–2. https://doi.org/10.1038/s41596-021-00655-6 ↩
-
Xie, X., Lokugamage, K. G., Zhang, X., Vu, M. N., Muruato, A. E., Menachery, V. D., & Shi, P.-Y. (2021). Engineering SARS-CoV-2 using a reverse genetic system. Nature Protocols, 16(3), 1761–1784. https://doi.org/10.1038/s41596-021-00491-8 ↩
-
Weaver, E. A., & Barry, M. A. (2008). Effects of Shielding Adenoviral Vectors with Polyethylene Glycol on Vector-Specific and Vaccine-Mediated Immune Responses. Human Gene Therapy, 19(12), 1369–1382. https://doi.org/10.1089/hum.2008.091 ↩
-
Arulanandam, R., Batenchuk, C., Varette, O., Zakaria, C., Garcia, V., Forbes, N. E., Davis, C., Krishnan, R., Karmacharya, R., Cox, J., Sinha, A., Babawy, A., Waite, K., Weinstein, E., Falls, T., Chen, A., Hamill, J., De Silva, N., Conrad, D. P., … Diallo, J.-S. (2015). Microtubule disruption synergizes with oncolytic virotherapy by inhibiting interferon translation and potentiating bystander killing. Nature Communications, 6(1), 6410. https://doi.org/10.1038/ncomms7410; Diallo, J.-S., Boeuf, F. L., Lai, F., Cox, J., Vaha-Koskela, M., Abdelbary, H., MacTavish, H., Waite, K., Falls, T., Wang, J., Brown, R., Blanchard, J. E., Brown, E. D., Kirn, D. H., Hiscott, J., Atkins, H., Lichty, B. D., & Bell, J. C. (2010). A High-throughput Pharmacoviral Approach Identifies Novel Oncolytic Virus Sensitizers. Molecular Therapy, 18(6), 1123–1129. https://doi.org/10.1038/mt.2010.67; Dornan, M. H., Krishnan, R., Macklin, A. M., Selman, M., El Sayes, N., Son, H. H., Davis, C., Chen, A., Keillor, K., Le, P. J., Moi, C., Ou, P., Pardin, C., Canez, C. R., Le Boeuf, F., Bell, J. C., Smith, J. C., Diallo, J.-S., & Boddy, C. N. (2016). First-in-class small molecule potentiators of cancer virotherapy. Scientific Reports, 6(1), 26786. https://doi.org/10.1038/srep26786 ↩
-
Kim, J., Hall, R., Lesniak, M., & Ahmed, A. (2015). Stem Cell-Based Cell Carrier for Targeted Oncolytic Virotherapy: Translational Opportunity and Open Questions. Viruses, 7(12), 6200–6217. https://doi.org/10.3390/v7122921; Power, A. T., Wang, J., Falls, T. J., Paterson, J. M., Parato, K. A., Lichty, B. D., Stojdl, D. F., Forsyth, P. A. J., Atkins, H., & Bell, J. C. (2007). Carrier Cell-based Delivery of an Oncolytic Virus Circumvents Antiviral Immunity. Molecular Therapy, 15(1), 123–130. https://doi.org/10.1038/sj.mt.6300039 ↩
-
Smith, S. M., Wachter, K., III, H. A. B., Schilsky, R. L., George, D. J., Peterson, D. E., Johnson, M. L., Markham, M. J., Mileham, K. F., Beg, M. S., Bendell, J. C., Dreicer, R., Keedy, V. L., Kimple, R. J., Knoll, M. A., LoConte, N., MacKay, H., Meisel, J. L., Moynihan, T. J., … Uzzo, R. (2021). Clinical Cancer Advances 2021: ASCO’s Report on Progress Against Cancer. Journal of Clinical Oncology. https://doi.org/10.1200/JCO.20.03420 ↩
-
Sadelain, M., Brentjens, R., & Rivière, I. (2013). The Basic Principles of Chimeric Antigen Receptor Design. Cancer Discovery, 3(4), 388–398. https://doi.org/10.1158/2159-8290.CD-12-0548 ↩